Beyond DNA: unlocking the secrets of the epigenome
A layer of regulatory information on top of DNA is proving to be as important as genes for development, health and sickness.
Why is a queen bee enormous compared to her worker bees? Why does one flower have petals that grow in a star-shape while another genetically identical flower forms petals in a mirror reflection? And how can two mice differ in color and shape even though they have the same DNA? In these cases and others, the two comparisons are genetically identical, possessing the same DNA code. So why does the same genetic code lead to very different outcomes?
Welcome to the epigenome. Many people are now familiar with the genome—a term used to denote the entirety of a person’s genetic code—but the epigenome, a lesser known, secondary layer of information that coats the genome, may be just as important in making us who we are.
This layer, made up of proteins and other molecules that bind onto the genes like tags, is proving to play a vital role in development, health and disease. Some have likened the epigenome to a symphony, where the DNA sequence is sheet music that can be expressed in vastly different ways through the direction of epigenomic regulators.
“The notes are identical but musicians can change the tempo or use a variety of instruments for different effects,” says Joseph Ecker, director of Salk’s Genomic Analysis Laboratory and codirector of California Institute for Regenerative Medicine (CIRM)’s The Center of Excellence for Stem Cell Genomics. “Likewise, these epigenetic factors along with other factors in cells each ‘play’ a role in how and when cells are to become heart tissue versus a liver, for example.”
The genome is constantly assailed by cellular molecules that temporarily turn genes on or off in response to the environment or in preparation for cell division. This is a stark contrast to more permanent changes like a mutation, where the gene itself is irreversibly changed.
The epigenome (whose precise definition can vary depending on who you talk to) can have both temporary and long-term effects on gene expression. It often consists of modifications to the genome that can persist for a time but are still ultimately reversible—able to fall off and leave the genes as they were.
One intriguing aspect of the epigenome is that epigenetic changes can be heritable, passed on from cell to cell as they divide and sometimes from parent to offspring. The epigenome may be affected by diet, activities like exercise or smoking, development and environment, suggesting that your lifestyle—and thus, epigenome—could have implications not just for you but also for your potential children or even grandchildren. Studies in mice, for example, suggest that how attentive a mother is to her pups after birth affects their epigenome, which later dictates levels of hormones and stress they experience as adults. The epigenome may also explain why some humans born in stressful or traumatic environments experience higher levels of sickness later in life, even in a safe and healthy setting.
“So much more research is necessary to determine if there is a causal link between cases where epigenetic variation has been observed and any health, diet or disease associations,” says Ecker, whose group participates in the NIH Roadmap Epigenomics Mapping and ENCODE (ENCyclopedia Of DNA Elements) Consortium. Both are efforts to map the epigenome and its intricate workings to understand how it influences the cell in development and how it changes in disease. More and more, scientists are showing that certain epigenomic changes can have a profound impact on everything ranging from cancer to diabetes to mental disorders. Scientists are banking on the idea that learning how to read—and manipulate—the epigenome may help us unlock entirely new suites of treatments.
MAPPING THE MUSIC
In the early 2000s, the announcement of the completion of the Human Genome Project led to unprecedented insights into how genetics influence health and disease. While fully sequencing the human genome promised to move us closer to personalized medicine that could individualize treatments to better cure disease, it turned out that mapping out the entire sequence of adenine, thymine, cytosine and guanine (A, T, C and G) pairs provides only a piece of the picture. A next step is to chart the epigenome—one component of which is the pattern of chemical tags called methyl groups that stud DNA to influence whether or not genes are activated. (See sidebar, page 16, for ways the genome can be modified.)
To explain how the epigenome works, some have likened it to a symphony: the sheet music (genome) is the same, but can be expressed in vastly different ways depending on the group of players and their instruments (epigenome).
What do these methyl tags do exactly? These branched molecules of carbon and hydrogen typically are attached to cytosine (the “C” of DNA base pairs) and tell other proteins to go away—or, in some cases, to gather—turning the attached gene off or on. Acting as on/off switches or stop-and-go signals, millions of methyl tags pepper the genomes of your cells.
Ecker first became intrigued by the epigenome while studying Arabidopsis thaliana, a small flowering plant used for basic plant biology research. He and his colleagues wanted to know how many Arabidopsis genes were controlled by DNA methylation. But while methylation has been studied for decades, there was no good way to get a snapshot of all the methylation marks in a cell’s DNA. So Ecker created one.
His lab designed a method called MethylC-Seq, which entails several chemistry techniques that break DNA into small pieces to process them through high-speed computers and technology such as sequencers built by the San Diego-based company Illumina. The resulting data allows scientists to, for the first time, see and quantify those methyl marks on the 3-billion-base genome. “MethylC-Seq allows us to extract that information from the invisible (methylated cytosine) and make it visible,” says Ecker, who is also a Howard Hughes Medical Institute and Gordon and Betty Moore Foundation investigator.
MethylC-Seq displays this methylation in patches of yellow, letting Ecker’s team compare different cells in one organism or across organisms to search for links, for instance, between aberrant DNA methylation and disease. Ecker’s methods have led to the first maps of the epigenome for plants and also animal cells, including humans, which has shown evidence that methylation increases from birth to adulthood. His major publication in 2015 in Nature provided, for the first time, details into the epigenome of a variety of tissues from organ donors of different genders and ages.
WHAT’S IN A BRAIN?
In 2013, Ecker and his neuroscience collaborators Margarita Behrens and Terrence Sejnowski published work in the journal Science showing something surprising. They had mapped the pattern of millions of methylation changes in the brain’s frontal cortex using human tissue. Methylation usually occurs on the DNA base cytosine (“C”) when it is adjacent to guanine (“G”). This epigenomic map of a healthy human brain revealed a type of methylation not normally seen in the body: methyl tags in neuronal cells were binding to DNA in places other than C. His team found that this alternative methylation (called non-CG) starts at birth and increases throughout early adulthood. Ecker and others suspect that abnormal patterns of methylation of this type might be associated with mental disorders like schizophrenia, whose symptoms don’t manifest until early adulthood.
Human DNA in a single cell is enormously long-six feet-and folds with proteins into packages (chromatin) to fit within a nucleus.
“We found a major difference in the epigenome of neurons which is an accumulation of a new kind of methyl mark that we didn’t even know existed before in neurons,” says Behrens, a Salk senior staff scientist. “This non-CG methylation accumulates from birth through adolescence during a critical period of brain maturation when connections are being made.”
When it comes to the brain, other studies have shown that environmental factors—medicine, diet, age, stress and chemical exposure—can change methylation in brain cells. Ecker and others are examining this global epigenomic reconfiguration during brain development to uncover potential ties to mental disorder. In one study, they are examining the effects of viruses and chemical exposure in animal models to see how environment changes this new type of methylation in brains during development. In another, his team has begun to map the epigenome of neurons from those with Alzheimer’s.
“Even if these epigenetic events are not causative for these disorders, they can provide useful markers of disease progression,” Ecker says. “And because methylation and other epigenomic changes are in essence temporary, there may be ways to tweak the epigenomic code to help treat diseases of the brain and the body.”
THE SHAPE OF DNA
Salk Assistant Professor Julie Law focuses on another aspect of the epigenome: the complicated and cryptic realm of chromatin, which is the dynamic combination of proteins, small RNAs and other molecules that surround DNA and which plays key roles in controlling its expression and stability.
“We are trying to understand how you get different outcomes from the same DNA,” says Law. “Epigenetic modifications are one way in which you can achieve this diversity to the genome in a manner that doesn’t change the underlying DNA sequence. But what we don’t understand is how epigenetic modifications target different places in various cells and are recognized and translated into desired responses.”
Part of the answer may be found in an epigenome change called chromatin remodeling, where proteins alter how the DNA is stored. Human DNA in a single cell is enormously long—six feet—and folds with proteins into intricate packages (chromatin) to fit within a nucleus. Imagine rolling and storing sweaters and mothballs into sealed vacuum bags to both keep them safe and make room for storage. In a similar way, the packaging of the DNA begins with histone proteins, which act as spools to wind DNA. The histones, DNA and other proteins are then further packaged into larger chromatin. Like sweaters hanging in the front of a closet, genes on the outside of chromatin are more easily accessed and usable than those stored deeply away.
Proteins that sit on top of the chromatin and control its spatial arrangement play an integral role in what genes are used or not and, similar to methyl tags, can have grave effects on health and disease. Adding chemical markers (methyl tags and others) to histone proteins can result in rearranging the chromatin, dictating which genes are ignored and which are turned on.
To understand these epigenetic factors, Law uses the Arabidopsis plant that, unlike mammals, is more tolerant of changes to the epigenome. She’s turned her attention to several newly identified families of proteins involved with DNA packaging and gene expression, called chromatin-binding proteins. She is striving to determine the epigenetic marks recognized by these protein families as well as identify their interacting partners and their effects on gene expression. Although Law’s research utilizes a plant model, her findings will also hold lessons for human biology as many of the genes involved in adding or removing epigenetic marks are the same in plants as in mammals.
Graham McVicker, a Salk assistant professor who studies the human genome and chromatin, says, “It’s as if the genome is a big landscape and the cell can plunk down flags—DNA methylation marks or histone modifications—to indicate which parts of the landscape have specific functions.” McVicker, who aims to understand how differences in the genome sequence affect our cells at a molecular level, adds that the genomes of unrelated individuals only differ by about one base in every thousand and that most of these differences have no function. “One way to understand which genetic differences are important is to look for the ones that are associated with the expression of genes or changes in histone modifications,” he says. “The goal is to understand how genetic differences affect gene expression or epigenetics and ultimately how these differences contribute to human traits and diseases.”
UNTANGLING CANCER
Salk Professor Beverly Emerson drills into the epigenome’s mechanistic processes from another angle: cancer.
In the last few decades, researchers have shown that changes in the epigenome are tied to many types of cancer through projects like ENCODE or NIH’s Cancer Genome Atlas (TCGA) Network, which compares genomes and epigenomes of normal and cancerous cells. Despite these efforts, it is difficult to untangle causes from correlations when it comes to epigenetics and cancer.
“The cell has a whole constellation of proteins to read and decode its DNA. Epigenetics is an elaborate way to allow the DNA coding sequence to be read in a manner contingent upon physiological signals,” says Emerson, holder of Salk’s Edwin K. Hunter Chair. “But when a DNA base change or a mutation occurs, epigenetic changes can promote the overgrowth of cancer or suppress the immune system.”
The body’s cells undergo changes in signaling to their genomes all the time: when you eat, when you get sunburnt, when you feel certain emotions. Emerson’s team is trying to distinguish those normal changes of the epigenome versus cancer-causing changes that result from chronic stress to the cell.
“There are so many changes in a cancer cell that often you don’t know what the driver was,” says Emerson. “Cancerous cells are already massively epigenetically modified, but we want to know how epigenetic changes and alterations in transcriptional programming primes cells to become cancerous.”
If we can better understand protein complexes involved in chromatin remodeling and how mistakes in making specific regions of the genome more or less accessible leads to cancer, we can develop targeted treatments.
By studying normal breast tissue samples and observing how those cells respond to prolonged stress, she hopes to understand how cells turn into breast cancer. To do this, she examines how alterations in DNA methylation and the cells’ chromatin regulators lead to critical tumor suppressant genes becoming rapidly silenced.
“Our hypothesis is that such abnormal cells accumulate with time and age. They’ve changed their epigenomic programming and are more vulnerable to becoming cancerous,” says Emerson. “A gene can become permanently locked into a repressed state through methylation, which is a problem if the gene happens to be a tumor suppressor or related to immune function.”
One way in which a gene can get locked into a repressed state is by mutations to epigenetic modifiers themselves, which is increasingly observed in the sequencing of human cancers. Salk Assistant Professor Diana Hargreaves is examining one such target in cancer, an epigenetic regulator called the SWI/SNF complex, which unpacks and unwinds DNA from chromatin to alter gene accessibility. Many pieces or subunits of the SWI/SNF complex are mutated in cancer, including ARID1A, which is mutated in ovarian, bladder, lung and colorectal cancers. Hargreaves is exploring the different activities of the SWI/SNF complex in normal and disease settings in order to figure out how to target ARID1A mutant cancers.
“We now know that mutations in chromatin remodeling proteins, such as ARID1A, can shift the balance of epigenetic modifiers at key tumor suppressor genes, leading to changes in histone modifications and gene accessibility that repress these genes. In the context of growth signals, this can rapidly lead to cancer,” says Hargreaves. “If we can better understand how mutations in protein complexes involved in chromatin remodeling prime the cell to become cancerous, we can begin to develop targeted treatments for SWI/SNF mutant and potentially other cancers.”
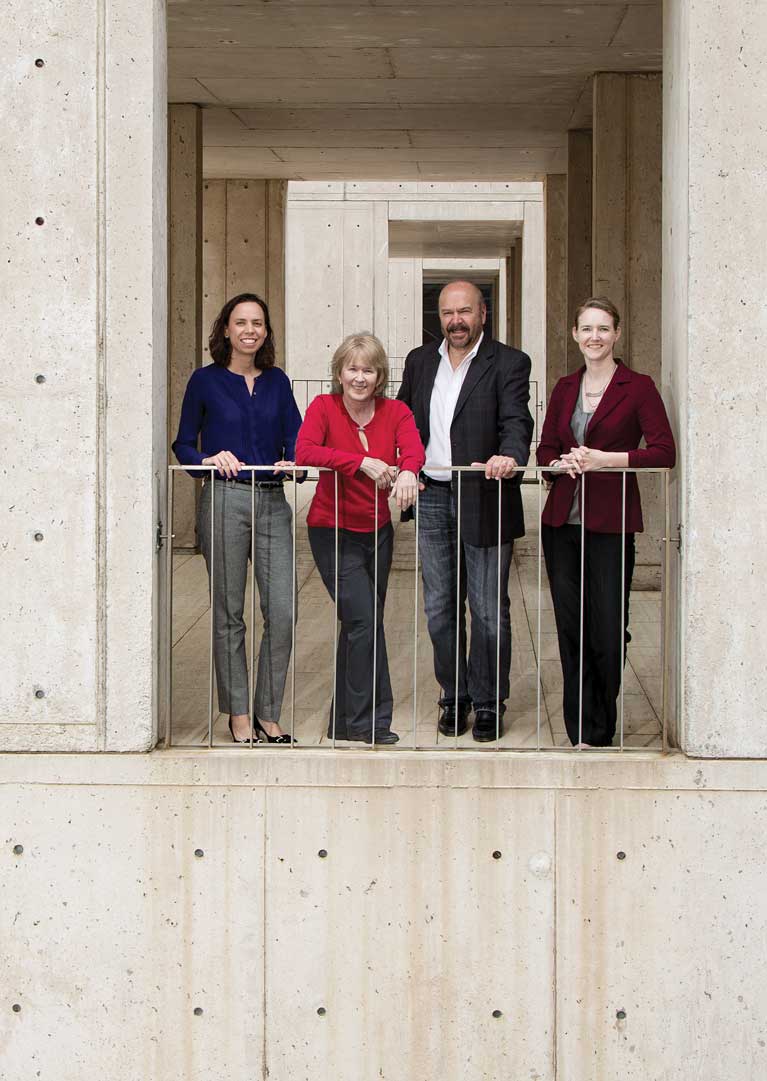
Researchers are already testing and applying drugs that target the epigenome by stripping away methylation in hopes of removing the tags that have silenced tumor suppressant genes. (One example is the drug temozolomide, used for treating brain tumors called glioblastoma.) However, there are some obstacles: such a treatment would strip away methylation on the rest of the genome, potentially leading to additional issues. Secondly, methylation is only one aspect of the epigenome; proteins that regulate chromatin are also often mutated in cancer. Nevertheless, refining ways to target methyl tags and other epigenetic regulators could be a boon for treatments in part because the epigenome is more reversible and malleable than say, a genetic mutation.
CHARTING THE EPIGENOME’S REACH
So far, researchers have shown that some types of modifications to histone proteins, cytosine methylation and molecules called small RNAs can be inherited in mice, flies and plants, for example, but it’s unclear to what extent epigenetics is inherited in people. “Just as everything—disease, obesity, depression—used to be blamed on the genome, now you will see all sorts of claims about the epigenome and I would not believe most of it,” Ecker says. “What I would say is that we’re really just at the beginning of understanding the epigenome and heritability but if there is the possibility that your diet or environment could affect your children or grandchildren, that will be important to know and exploring the molecular mechanisms using model systems may help us to understand these phenomena better.”
Aside from exploring methods of inheritability, Ecker and others are now making a slew of maps of normal and disease states to generate comprehensive epigenomic references for everything from neurodegenerative and developmental disorders to metabolic diseases like diabetes as well as cancer. In one study, Ecker and Salk Professor Ronald Evans are studying how exercise might affect your epigenome. In another effort recently funded by the NIH, Ecker is researching whether viruses can act on the epigenome during development to cause autism.
“At Salk, we have scientists interested in understanding epigenetics related to cancer, neuroscience, regenerative medicine and other fields, as well as researchers who have the potential to make small molecule drugs to target the epigenome,” says Ecker. “We’re really at the very beginning and it’s the interplay of these areas of specialties that is going to advance the science and help us understand how the epigenome is affected by development, nutrition, disease and aging.”
Support a legacy where cures begin.
Featured Stories
Beyond DNA: Unlocking the Secrets of the EpigenomeA layer of regulatory information on top of DNA is proving to be as important as genes for development, health and sickness.
Tapping our Immune System’s SuperpowerWe all have a superhero—or supervillian—inside our bodies. It’s called our immune system. Every day, a healthy immune system repels a host of adversaries—bacteria, viruses, parasites—you name it!
All about town with Pablo HollsteinGrowing up in Quito, Ecuador, Pablo Hollstein was passionate—and precise —about science from an early age.