FRONTIERS The Aging Puzzle comes together
What is aging?
That question is more complex than it may appear. On an intuitive level, it’s our bodies becoming less resilient. Strenuous exercise, minor muscle strains, late nights out with friends—we just can’t bounce back like we used to.
Aging has even greater ramifications for disease. The risk of developing cancers, neurodegenerative conditions, heart disease and diabetes all increase as we get older. One current example is COVID-19, which tends to generate only mild symptoms in young people, while many over 65 are hospitalized.
We recognize, from personal experience, what aging does to our bodies; we just don’t fully understand why. Something is happening at the molecular, cellular, tissue and organ levels to make our bodies less resilient and more prone to disease.
“We lack the mechanistic scientific understanding of what health really means at different stages of life,” says Martin Hetzer, vice president/chief science officer and professor. “Health at age 20 means something different from when we are 40, 60 or 80. But what does that look like biologically? How do these systems change over time?”
If aging is the critical switch that sets the stage for multiple conditions, scientists want to understand what happens if we adjust that switch to increase health span—the number of years we are free from age-related decline and disease. By restoring some of the body’s youthful resilience, we could revolutionize public health, the way scientists did with vaccines and antibiotics.
“Aging is the biggest risk factor for most diseases, but our health care system tends to manage these one at a time,” says Gerald Shadel, professor and holder of the Audrey Geisel Chair in Biomedical Science. “We go to the cardiologist if we have a heart problem, a rheumatologist if we have arthritis. But, if we could manage aging itself, push these age-related conditions down the road, we could dramatically reduce the overall burden of disease on society and increase individual health span.”
Understanding Aging’s Many Pieces
Aging is multifaceted, making it the perfect puzzle for Salk researchers to piece together. The Institute is approaching aging from multiple, converging angles: genomics, epigenetics, neurology, immunology, cell biology, data science and more. Understanding how these mechanisms interact may reveal how human biology changes with age and how that makes our bodies more vulnerable to disease.
Inflammation’s Central Role
“Inflammation is a hallmark of aging,” says Susan Kaech, professor and director of the NOMIS Center for Immunobiology and Microbial Pathogenesis. “But when we say inflammation, what molecules are we talking about and what are they doing? Defining the elements of inflammation most associated with age-related pathologies is a cornerstone of aging research right now.”
Chronic inflammation, which often happens when the immune system goes on permanent alert, can generate body aches, fatigue, insomnia and other issues, and is often linked to biological stresses, such as repeated infections. Consider our emotional response to a near-miss on the freeway: stress floods our bodies. Cells have their own stress responses, and they may leave a mark.
“Maybe you had a pulmonary infection, like COVID-19, which caused inflammation in your lungs,” says Kaech.
“Those cells have acquired a history of seeing inflammation; they are basically imprinted by that inflammatory exposure. Now, take that over the course of a lifetime, with multiple infections. Cells may remember that history, making them hyper-prepared for the next infection. We want to understand the long-term impact that could have on tissue health.”
This is part of a larger effort to determine how cells and tissues get remodeled with age. In another immune-related example, T cells infiltrate the brain, though nobody is quite sure what they do there.
“T cells can live in the brain long-term after an infection,” says Kaech. “We believe this is an evolutionary process to seed the body with long-lived memory cells that could respond to a second infection and protect us.”
Kaech and colleagues wonder if these and other infection-related changes can have long-term consequences, contributing to cellular changes that make the body less resilient against Alzheimer’s disease and other conditions.
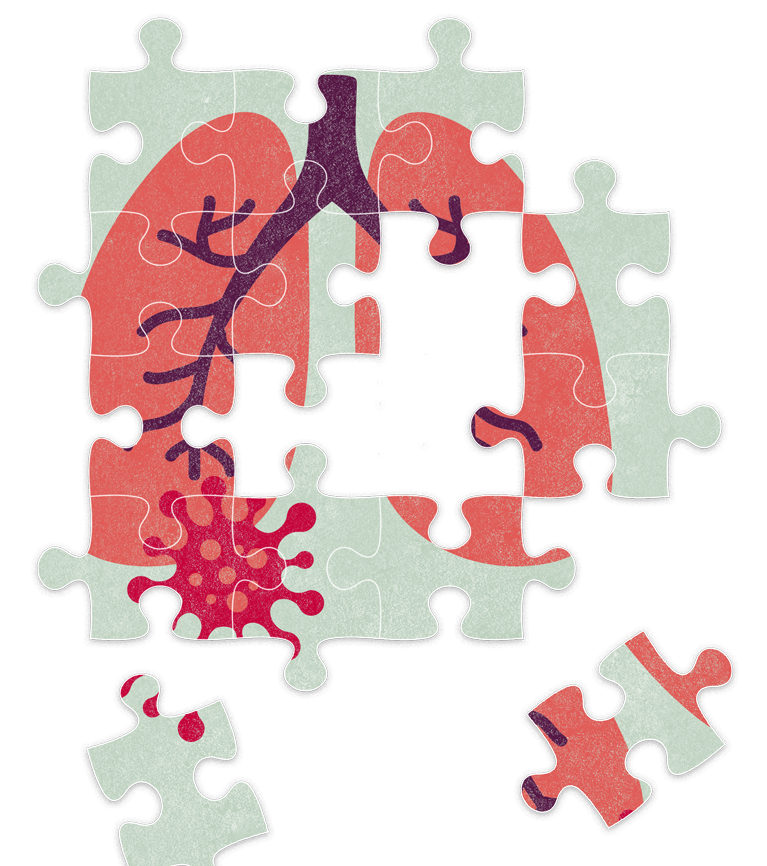
“Maybe you had a pulmonary infection, like COVID, which caused inflammation in your lungs. Those cells have acquired a history of seeing inflammation; they are basically imprinted by that inflammatory exposure. Now, take that over the course of a lifetime, with multiple infections. We want to understand the long-term impact that could have on health.” – Susan Kaech
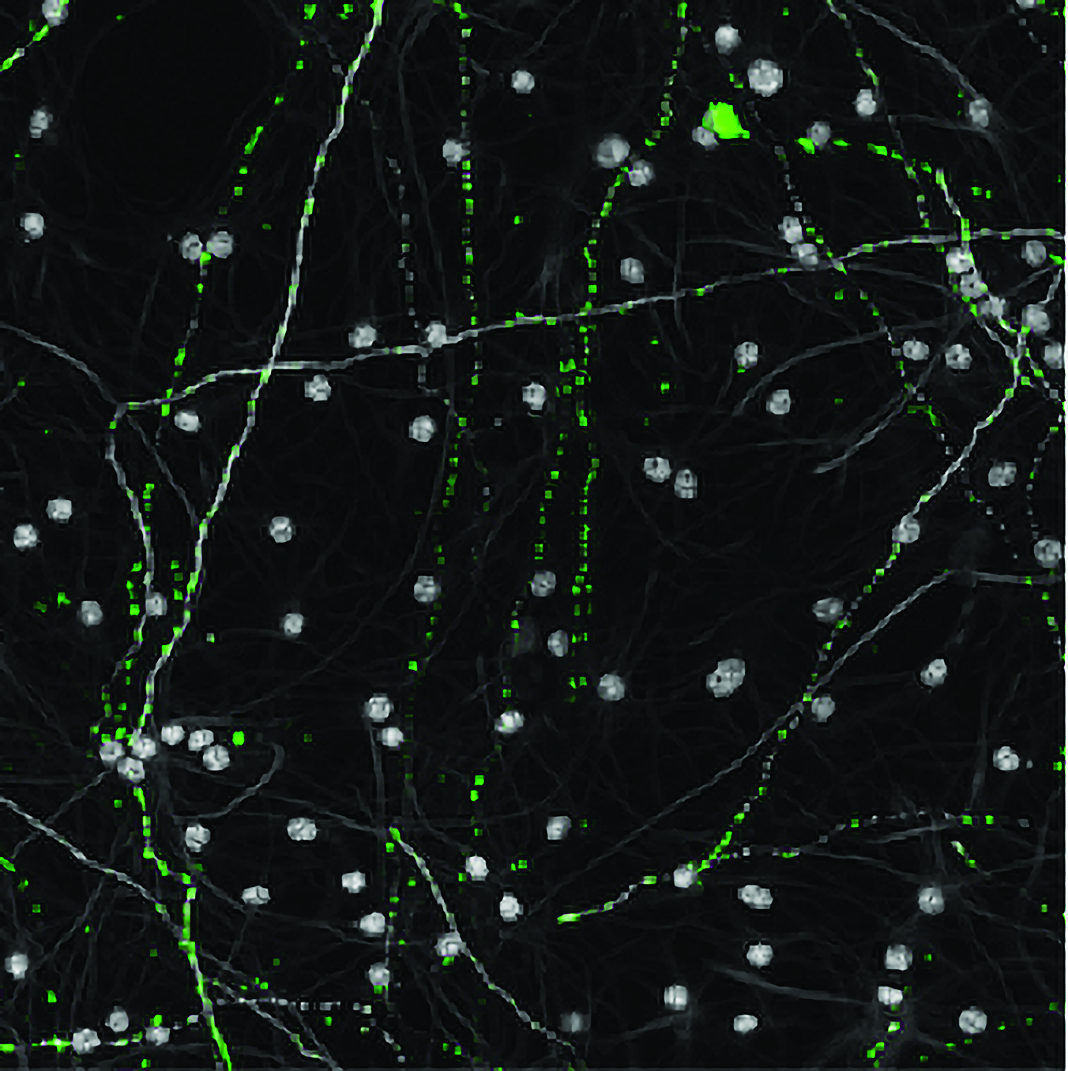
Aged mitochondria (green) in old neurons (gray) appear mostly as small punctate dots rather than a large interconnected network.
Energy Consumption
Neurons consume a great deal of energy—using ten times more cellular energy packets, adenosine triphosphate (ATP), than other cells, on average. Most of this energy is consumed at the synapse, the critical part of the neuron that enables new memories to form. Understanding this energy economy is vital to dissect neuronal aging and perhaps the memory loss associated with Alzheimer’s.
The problem starts with mitochondria, the cellular factories that manufacture ATP. As we age, mitochondria become less efficient and generate less energy. To make matters worse, aging mitochondria give off reactive oxygen species, which can damage the neuron and its DNA. Repairing this damage is, in itself, an energy-consuming process, which sets up a vicious cycle: aging mitochondria damage DNA; DNA repair mechanisms require more energy to fix the damage; stressed mitochondria fail to meet those energy needs.
“We’re very excited to be able to begin to understand the connection between mitochondrial aging and age-related cognitive decline,” says John Reynolds, professor in the Systems Neurobiology Laboratory and Fiona and Sanjay Jha Chair in Neuroscience. “Now, we can visualize the mitochondria in neurons in a dish and see how they change with age. We’re finding that animals showing age-related cognitive decline have mitochondrial aging signatures not present in healthy animals. With this approach, we’re beginning to get a handle on how this system may be driving vulnerability to Alzheimer’s disease.”
Once again, the story circles back to inflammation. Mitochondria are relics from a symbiotic union between bacteria and other single-celled organisms around 1.4 billion years ago. They make multicellular life possible but, because they’re biological hitchhikers, mitochondria have their own DNA (mtDNA). The relationship works great, mostly, but sometimes mitochondria break down and release mtDNA into the cellular environment. This “foreign” DNA is treated like an invader—a bacterium or virus. Inflammation ensues.
“We’ve connected mitochondria and mtDNA to the inflammation axis and are trying to drill down on the relevance of this to aging,” says Shadel. “If we can prevent that release of inflammatory molecules from mitochondria, we predict it could have a health-span-promoting effect.”
The Rewards of Circadian Optimization
The body is often likened to a machine, but that comparison is not entirely accurate. For example, whether you start a car at 8 a.m. or 8 p.m., its performance will hardly vary. But our bodies are governed by a 24-hour clock. How we perform varies significantly with the timing.
Circadian rhythms influence every tissue in our bodies and have a profound impact on metabolism, sleep, digestive function, mental acuity and much more.
“During the daytime, circadian rhythms delineate the timing of peak performance in various organs,” says Satchin Panda, professor in the Regulatory Biology Laboratory. “But they also govern repair and reset at night. Only when we have this performance and repair, on a daily basis, can we sustain better health.”
It’s easy to feel we can ignore these cycles—after all, we have artificial light and lots of great coffee—but the body needs to rejuvenate at night, whether we like it or not. Without this opportunity to refresh, the liver accumulates fat, which can lead to insulin resistance, diabetes and fatty liver disease. Denying kidney and heart cells their repair cycles can lead to high blood pressure.
“Every organ has its own clock, which means every organ needs to ‘sleep,’” says Panda. “But it’s not sleep they need so much as time without food. You cannot repair a highway while traffic is flowing, and you cannot repair your gut lining if you just ate.”
Panda’s research evokes a concept called circadian optimization. The entire genome is programmed to go through these up and down cycles at different times of the day in different cells. By acknowledging circadian rhythms, and adjusting our lifestyles to collaborate with them, we can empower this repair cycle at night and amplify biological performance by day.
“We suggest that people not eat immediately after they wake up, for one to two hours,” says Panda, “when the hormonal balance is flipping from nighttime repair to daytime functionality. Then eat during a 10 hour window, maximum 12 hours.”
Panda believes circadian rhythms could be harnessed as a therapeutic strategy. He points to emerging research, from his lab and others, that suggests drugs targeting our body clocks could help treat certain cancers, neurodegenerative conditions and other diseases.
“We think the circadian rhythm theory of health will have a tremendous public health impact,” says Panda. “By changing a few simple habits, we could reduce the risk of diabetes, cardiovascular disease, colon cancer and chronic inflammation and even accelerate healing.”
Telomeres and Aging
Telomeres are small DNA stretches at the end of each chromosome that play an oversized role in determining whether cells can divide indefinitely or not. In most human cells, telomeres are whittled away after each cell division. Early in life, this shortening process has little impact on human health, but once telomeres are too short, cells stop dividing and enter a state of irreversible arrest, which can be highly inflammatory.
Jan Karlseder, professor in the Molecular and Cell Biology Laboratory, has spent years investigating how these truncated telomeres send a DNA damage signal that prevents cancer cells from becoming immortal, as well as keeping their genome stable.
“Until recently, I had not considered that shortened telomeres send signals far beyond the ends of chromosomes,” says Karlseder, “but my lab recently discovered that this process activates inflammation pathways via the mitochondria, which surprised all of us.”
Karlseder’s group is now working with Gerald Shadel’s lab to decipher this cross talk between telomeres and mitochondria, as part of Salk’s focus on understanding the synergy between different cellular systems during the aging process.
But not all human cells undergo telomere shortening at the same rate. In adult stem cells, a telomere-dedicated machine called telomerase intervenes to slow down the shortening process Vicki Lundblad, also a professor in the Molecular and Cell Biology Laboratory, studies how telomerase functions inside cells, which has led to an unexpected discovery.
“It had been widely assumed that telomerase acts only after chromosomes have been fully replicated, just prior to cell division,” says Lundblad. “Much to our surprise, we discovered that telomerase instead prefers to act at sites of chromosome replication failures.”
This unexpected result has revealed a new network of genes that control telomerase access to these sites—providing potential targets for further aging research.
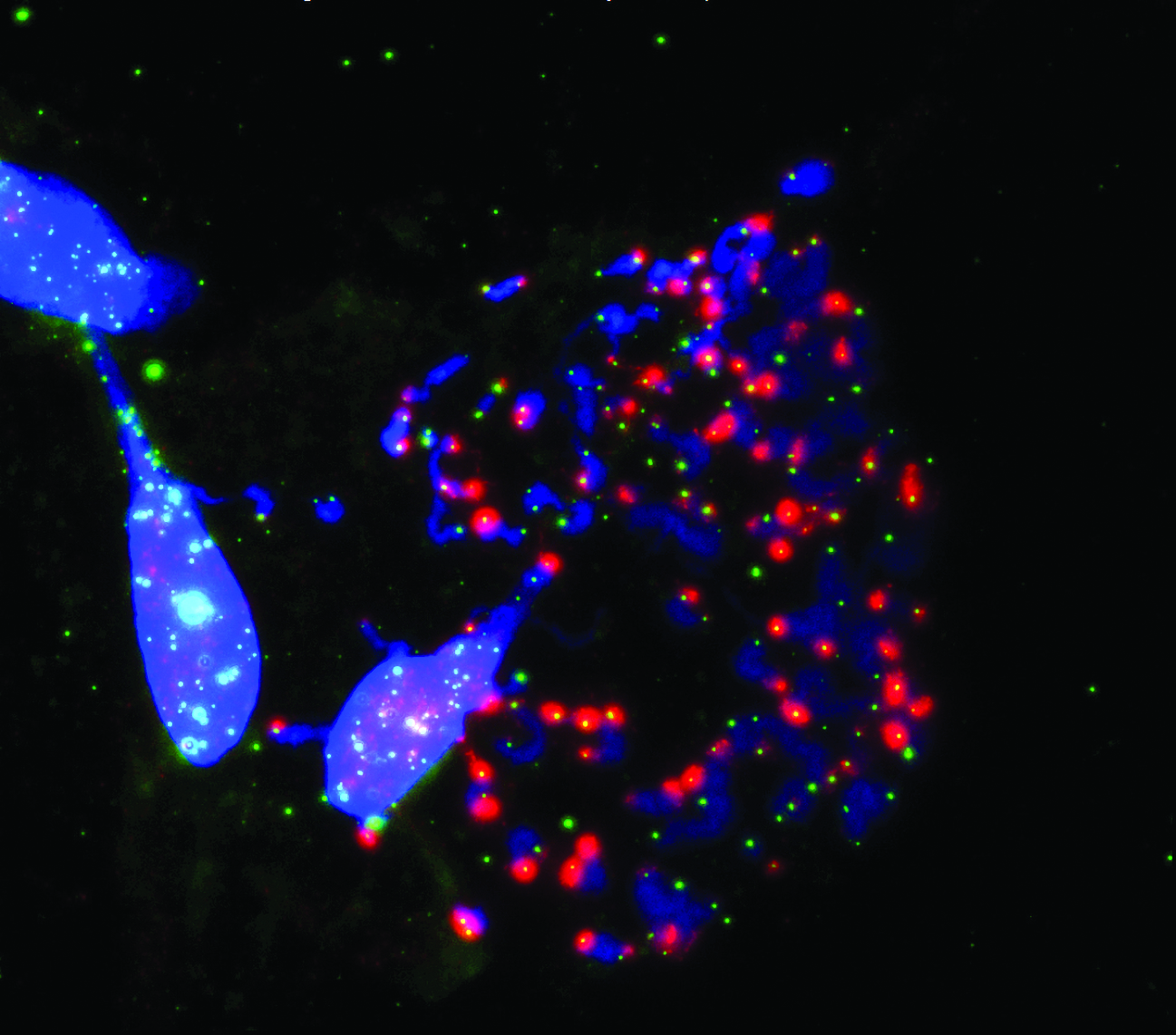
Salk researchers show how the disabled protection of ends of chromosomes (blue) called telomeres (green) during cell division (mitosis) prompts cell death.
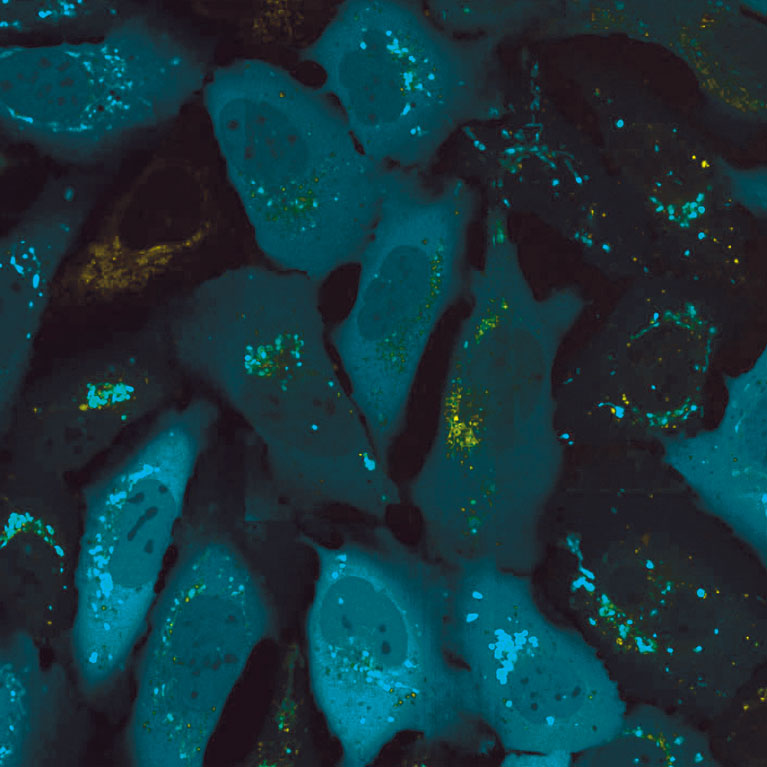
Parkin (above) is an autophagy gene that helps regulate the cellular recycling system. Parkin is also linked to early onset Parkinson’s disease.
Cellular Garbage Disposals
Like Karlseder, Reuben Shaw has long studied mechanisms associated with cancer. He directs Salk’s NCI-designated Cancer Center and has spent years investigating a protein pathway called AMPK, which helps govern how cells respond to scarce nutrition—a major issue for tumors. These studies helped him unwind how metabolism can be weaponized against cancer, but they also led in an unforeseen direction—neurodegenerative disease.
The key has been understanding what AMPK, and associated proteins, do outside of disease: their day jobs. The Shaw lab found AMPK was talking to another protein called ULK1, which regulates a cellular recycling system called autophagy. It turns out ULK1 modulates an autophagy gene called Parkin, which is closely linked to early onset Parkinson’s disease, as well as recycling mitochondria.
“In three biochemical moves we went from a cancer suppressing enzyme, called LKB1, which is mutated in around 20 percent of lung cancers, to AMPK to ULK1 to Parkin, which is directing the cell’s recycling center and playing a role in preventing neurodegenerative disease,” says Shaw.
Normally, this pathway gets turned on when cellular machinery goes awry to get it back on track. This protective effect could also be harnessed to control age-related diseases.
“The AMPK pathway has potent anti-cancer properties,” says Shaw, “as well as anti-diabetes properties and, at least in the case of Parkinson’s, anti-neurodegenerative disease properties.”
Putting the Pieces Together
Inflammation, telomeres, mitochondria, and autophagy all play individual roles in aging. But how do these elements work in unison?
“We have the parts list,” says Hetzer. “We basically know how a cell works. Now, we can put this together and look at more than just one or two components—we can look at the entire system.”
In late 2018, the Salk Institute received a $19.2 million grant from the American Heart Association-Allen Initiative to study mechanisms that make the brain susceptible to Alzheimer’s disease and cognitive decline and develop new therapies.
The grant is directly funding research in nine laboratories, creating a collaborative consortium to identify the mechanisms that drive neural aging and find creative ways to counteract them.
“We all study cellular and molecular hallmarks of aging,” says Shadel. “We know that mitochondria, inflammation, etc., are all important, and we each study these things in our own ways. But this new initiative allows us to collaboratively investigate the interactions between different aging hallmarks and get the big picture of aging in the brain.”
Led by Salk President Rusty Gage, this effort leverages the Institute’s intellectual firepower in metabolism, immunology, inflammation, genetics, epigenetics and other areas to better understand how we can make the brain more resilient as it ages.
“It’s not just finding a cause or cure for Alzheimer’s disease,” says Gage, “it’s fortifying a healthy brain to make us less susceptible to disease.”
But before this large research collaboration could fully investigate the aging brain, and align their studies, they needed a robust model to test their ideas.
How to Study the Brain
Scientists are always looking for better ways to model biology. By changing an input, such as shutting down a gene, they can see how the model responds.
But studying aging in the brain is tricky. Researchers need models that are age-equivalent to the patients they are modeling, as well as replicating the neural environment at different ages. Fortunately, the Gage lab has developed two key tools—age-equivalent neurons and 3D brain organoids—that help solve both problems.
The technique starts with skin cells reverted into induced pluripotent stem cells (iPSCs), which can then be differentiated into virtually any cell type. However, when cells revert to iPSCs, their age resets to zero. Gage discovered a way to maintain the cell’s original age, so the neurons being studied reflect the age of the donor.
By maintaining this cellular time stamp, researchers throughout the AHA-Allen collaboration can do in-depth studies to better understand how various cellular characteristics change with age.
This model is a big deal, elevating research throughout the Institute. Whether scientists are studying immune cells, inflammatory pathways, telomeres, mitochondria, or other components, the Gage lab’s brain organoids give them tremendous opportunities to assess the aging brain. Equally important, the model gives collaborators common ground to investigate how these different functions play off each other.
“Understanding aging is more complicated than saying there’s a decrease in mitochondrial function or a shortening of telomeres or increased inflammation,” says Gage. “It is the interaction between all of them, creating a brain environment that is conducive to disease.”
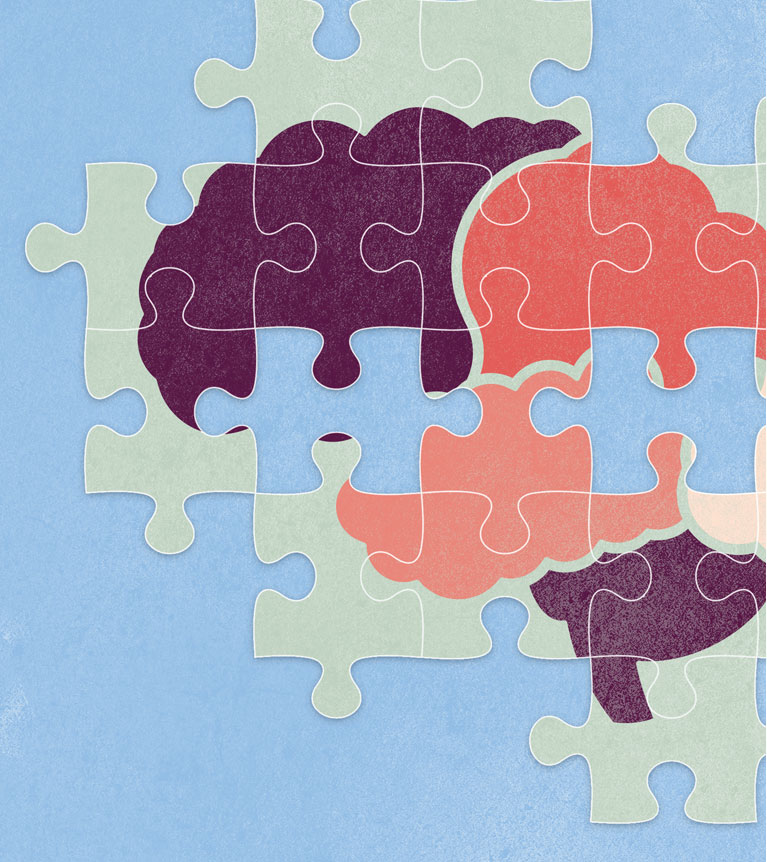
“We all study hallmarks of aging.We know that mitochondria, inflammation, etc., are all important, and we study these things in our own ways. But this allows us to step back and investigate the interactions between different mechanisms and get the big picture of aging in the brain.” – Gerald Shadel
Genomic On/Off Switches
Many of the mechanisms described throughout this article are influenced by the epigenome, the molecules that control which genes get turned on and off. Epigenetic markers often change based on environmental inputs over time, making them critical to understanding aging.
Joseph Ecker is a professor in the Plant Molecular and Cellular Biology Laboratory and an expert on the epigenome. Epigenetic markers are ubiquitous and so is Ecker, collaborating with multiple labs in the AHA-Allen project, as well as the Cancer Center and the Harnessing Plants Initiative.
Ecker is working closely with Gage and others to understand the epigenetic profiles in aging brains. Like inflammation, mitochondrial dysfunction, immune cell migration and other mechanisms, epigenetic changes could be making older brains more susceptible to disease.
“We have a nice collaboration with Rusty’s lab to go deep into single-cell profiles and ask how much of the aging signature is epigenetic,” says Ecker. ”Can we see differences between aging in Alzheimer’s versus in individuals without Alzheimer’s? The results are already looking pretty interesting.”
Ecker, a Howard Hughes Medical Institute investigator, believes they are getting closer to understanding how epigenetics, and thus gene expression, change in the brain over time, insights that could eventually power new interventions.
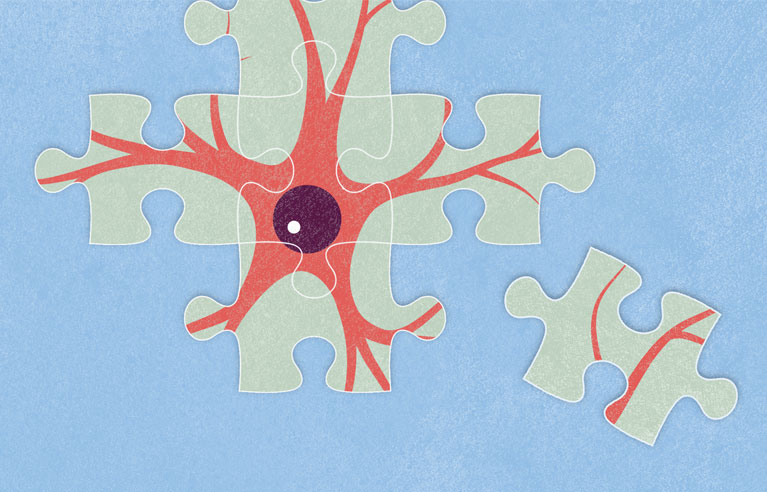
Astrocytes, star-shaped brain cells that do a little bit of everything, may hold major clues to making the brain environment more resilient against disease.
How the Brain Remodels with Age
Neurons are the popular kids in the brain, getting the most attention from researchers. But they don’t act alone.
“If we want to come up with new therapies for developmental disorders, neurodegenerative diseases and aging, we need to think about the many other types of cells in the brain, not just neurons,” says Nicola Allen, associate professor in the Molecular Neurobiology Laboratory.
Allen is particularly focused on astrocytes, star-shaped brain cells that do a little bit of everything: maintaining neurons, regulating blood flow, providing neurons with energy sources. Astrocytes are brain generalists.
The Allen lab was studying astrocytes in development when an interesting question crept into their discussions. “We know astrocytes are incredibly important during development,” says Allen, “but what about the rest of life? Do the molecular properties of these cells change with age and, if so, what do those changes mean? We’re not thinking aging astrocytes trigger Alzheimer’s so much as they change the neural environment, allowing neurodegenerative disease to happen.”
Allen wants to know what aging has to do with disease. Alzheimer’s, ALS and Parkinson’s all have genetic components, but they generally appear later in life. Something is changing in the brain that allows these diseases to gain traction.
Astrocytes may hold major clues. The Allen lab is investigating whether they can reverse some of these changes and make the brain environment more resilient. However, the intervention must be just right.
“We don’t want to send these cells all the way back to the development stage; that’s a very different brain environment,” says Allen. “But perhaps we can send them back from aging to an adult status. We could get rid of the inflammatory pathways actively damaging neurons, but still keep everything stable.”
The Opposite of Aging
Induced pluripotent stem cells (iPSCs) were a brilliant breakthrough by Japanese scientist Shinya Yamanaka , for which he won the Nobel Prize in 2012. By exposing adult skin cells to four types of proteins, called transcription factors, he reprogrammed them epigenetically, nudging them into an immature state, similar to embryonic stem cells. From there, he could differentiate the iPSCs into virtually any cell type, while keeping the genome from the original cell.
This process intrigued Juan Carlos Izpisua Belmonte, professor in the Gene Expression Laboratory. His lab showed that during the reprogramming process, cells lose the molecular characteristics of aging that normally accumulate over time. He wondered if this technique could be used to rejuvenate cells to make them healthier, rather than reprogramming them all the way back to a pluripotent state.
“If we put these four factors in a live organ, for example a heart, we no longer have beating heart cells or cardiomyocytes,” says Izpisua Belmonte. “You convert heart cells into embryonic stem cells and the heart will stop beating, which is not what we want.”
To solve the problem, Izpisua Belmonte and colleagues brought time into the equation. Rather than bathing the cells in the four factors for a long time, they pulsed them for short periods. This made the cells de-differentiate, like Yamanaka’s. They just didn’t go all the way back to the starting line.
To test the beneficial effects of this process, Izpisua Belmonte used Yamanaka factors therapeutically in a mouse model of progeria, a rare disease that ages children prematurely. The mice would normally have lived around four months; however, this treatment extended their lives significantly.
Izpisua Belmonte is quick to point out that there are differences between premature and normal aging. This rejuvenation approach has now been tested in physiologically aging mice. His group has recently shown that it can also increase the capacity of some tissues, such as muscle, to regenerate and become functional again.
“We now have the ability to rejuvenate an organism in the lab,” says Izpisua Belmonte. “We need to better understand how this process happens so that the knowledge could be used in clinical settings in the future.”
The Power of Collaboration
Since the AHA-Allen grant, Salk has become a nexus for aging research, particularly neurological aging. In 2019, Ecker and three other researchers received a $12.9 million grant from the NIH BRAIN Initiative to illuminate how the brain works and what can go wrong to cause disease.
More recently, in late 2020, Shadel helped create a scientific consortium between Salk, UC San Diego and Sanford Burnham Prebys Medical Discovery Institute to establish the San Diego Nathan Shock Center (SD-NSC) of Excellence in the Basic Biology of Aging.
“The SD-NSC is this incredible collaboration we just started that is studying the basic biology of aging and promoting aging research,” says Shadel. “Specifically, we are focusing on how and why different cells and tissues age at different rates and how this variability contributes to how individuals age and become susceptible to certain diseases.”
In 2021, John Reynolds and colleagues received a $1.2 million grant from the Larry L. Hillblom Foundation to examine aging and disease. “This grant came out of collaborative efforts made possible by the American Heart Association and the Allen Institute support,” says Reynolds. “This has really opened up the opportunity for me as a cognitive neuroscientist to work with some fantastic cell biologists and others who have been studying aging in different ways. It brings the science together.”
From Research to Potential Therapies
The AHA-Allen grant, and other support, is producing insights into how the body ages that could lead to new therapeutic approaches to mitigate it. For example, Senior Staff Scientist Pamela Maher (click here for “Insights” feature) and colleagues have shown two potential drugs slow aging in a mouse model of Alzheimer’s disease.
Martin Hetzer was one of the first to show that different tissues naturally age at different rates, a process called age mosaicism. And new machine-learning techniques may help predict how quickly an individual’s cells could age, which might encourage lifestyle changes to potentially mitigate some of these effects. The Hetzer lab has identified molecular targets that could slow blood vessel aging. This is particularly important for neurological diseases, as hard, leaky vessels may play a significant role.
AHA-Allen and other funding has supercharged Salk’s natural collaborative approach. While different scientists bring their own knowledge to the table, there is tremendous cross talk between disciplines. This convergence of expertise is providing new insights that could illuminate the aging process and boost resilience against multiple diseases.
“We all study different things, but our work comes together with aging,” says Shaw. “It’s like that proverb of the blind men and the elephant. One investigates the trunk, another the tail, another the legs—so their descriptions are all different. But what if these men could suddenly see? Imagine their amazement at discovering they had been talking about the same thing all along.”
Support a legacy where cures begin.
Featured Stories
The aging puzzle comes togetherAging is a complex puzzle, but by applying a collaborative, multidisciplinary approach, Salk scientists are putting its many pieces together.
Dmitry Lyumkis – A passion for problem solvingAssistant Professor Dmitry Lyumkis discusses what he loves about data and the scientific process, and which places inspire him outside the lab.
Pamela Maher – Seeking treatments for Alzheimer’s diseaseFrom having a large garden to investigating compounds that plants make, Staff Scientist Pam Maher talks about how plants inspire her to find treatments for Alzheimer’s disease.
Rajasree Kalagiri – Bound to phosphohistidineRajasree Kalagiri shares the serendipitous steps along her journey of scientific discovery from southern India to Southern California.